Authors: R. Bernstein, P. Cooper
Reference: 1307.5787 (Phys. Rept. 532 (2013) 27)
Not all searches for new physics involve colliding protons at the the highest human-made energies. An alternate approach is to look for deviations in ultra-rare events at low energies. These deviations may be the quantum footprints of new, much heavier particles. In this bite, we’ll focus on the decay of a muon to an electron in the presence of a heavy atom.
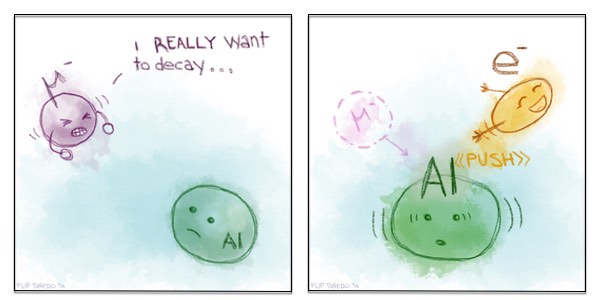
The muon is a heavy version of the electron.There are a few properties that make muons nice systems for precision measurements:
- They’re easy to produce. When you smash protons into a dense target, like tungsten, you get lots of light hadrons—among them, the charged pions. These charged pions decay into muons, which one can then collect by bending their trajectories with magnetic fields. (Puzzle: why don’t pions decay into electrons? Answer below.)
- They can replace electrons in atoms. If you point this beam of muons into a target, then some of the muons will replace electrons in the target’s atoms. This is very nice because these “muonic atoms” are described by non-relativistic quantum mechanics with the electron mass replaced with ~100 MeV. (Muonic hydrogen was previous mentioned in this bite on the proton radius problem.)
- They decay, and the decay products always include an electron that can be detected. In vacuum it will decay into an electron and two neutrinos through the weak force, analogous to beta decay.
- These decays are sensitive to virtual effects. You don’t need to directly create a new particle in order to see its effects. Potential new particles are constrained to be very heavy to explain their non-observation at the LHC. However, even these heavy particles can leave an imprint on muon decay through ‘virtual effects’ according (roughly) to the Heisenberg uncertainty principle: you can quantum mechanically violate energy conservation, but only for very short times.

One should be surprised that muon conversion is even possible. The process $latex \mu \to e$ cannot occur in vacuum because it cannot simultaneously conserve energy and momentum. (Puzzle: why is this true? Answer below.) However, this process is allowed in the presence of a heavy nucleus that can absorb the additional momentum, as shown in the comic at the top of this post.
Muon conversion experiments exploit this by forming muonic atoms in the 1s state and waiting for the muon to convert into an electron which can then be detected. The upside is that all electrons from conversion have a fixed energy because they all come from the same initial state: 1s muonic aluminum at rest in the lab frame. This is in contrast with more common muon decay modes which involve two neutrinos and an electron; because this is a multibody final state, there is a smooth distribution of electron energies. This feature allows physicists to distinguish between the $latex \mu \to e$ conversion versus the more frequent muon decay $latex \mu \to e \nu_\mu \bar \nu_e$ in orbit or muon capture by the nucleus (similar to electron capture).
The Standard Model prediction for this rate is miniscule—it’s weighted by powers of the neutrino to the W boson mass ratio (Puzzle: how does one see this? Answer below.). In fact, the current experimental bound on muon conversion comes from the Sindrum II experiment looking at muonic gold which constrains the relative rate of muon conversion to muon capture by the gold nucleus to be less than $latex 7 \times 10^{-13}$. This, in turn, constrains models of new physics that predict some level of charged lepton flavor violation—that is, processes that change the flavor of a charged lepton, say going from muons to electrons.
The plot on the right shows the energy scales that are indirectly probed by upcoming muonic aluminum experiments: the Mu2e experiment at Fermilab and the COMET experiment at J-PARC. The blue lines show bounds from another rare muon decay: muons decaying into an electron and photon. The black solid lines show the reach for muon conversion in muonic aluminum. The dashed lines correspond to different experimental sensitivities (capture rates for conversion, branching ratios for decay with a photon). Note that the energy scales probed can reach 1-10 PeV—that’s 1000-10,000 TeV—much higher than the energy scales direclty probed by the LHC! In this way, flavor experiments and high energy experiments are complimentary searches for new physics.
These “next generation” muon conversion experiments are currently under construction and promise to push the intensity frontier in conjunction with the LHC’s energy frontier.
Solutions to exercises:
- Why do pions decay into muons and not electrons? [Note: this requires some background in undergraduate-level particle physics.] One might expect that if a charged pion can decay into a muon and a neutrino, then it should also go into an electron and a neutrino. In fact, the latter should dominate since there’s much more phase space. However, the matrix element requires a virtual W boson exchange and thus depends on an [axial] vector current. The only vector available from the pion system is its 4-momentum. By momentum conservation this is $p_\pi = p_\mu + p_\nu$. The lepton momenta then contract with Dirac matrices on the leptonic current to give a dominant piece proportional to the lepton mass. Thus the amplitude for charged pion decay into a muon is much larger than the amplitude for decay into an electron.
- Why can’t a muon decay into an electron in vacuum? The process $latex \mu \to e$ cannot simultaneously conserve energy and momentum. This is simplest to see in the reference frame where the muon is at rest. Momentum conservation requires the electron to also be at rest. However, a particle has rest energy equal to its mass, but now there’s now way a muon at rest can pass on all of its energy to an electron at rest.
- Why is muon conversion in the Standard Model suppressed by the ration of the neutrino to W masses? This can be seen by drawing the Feynman diagram (fig below from 1401.6077). Flavor violation in the Standard Model requires a W boson. Because the W is much heavier than the muon, this must be virtual and appear only as an internal leg. Further, W‘s couple charged leptons to neutrinos, so there must also be a virtual neutrino. The evaluation of this diagram into an amplitude gives factors of the neutrino mass in the numerator (required for the fermion chirality flip) and the W mass in the denominator. For some details, see this post.
Further Reading:
- 1205.2671: Fundamental Physics at the Intensity Frontier (section 3.2.2)
- 1401.6077: Snowmass 2013 Report, Intensity Frontier chapter
Flip Tanedo
Latest posts by Flip Tanedo (see all)
- The Delirium over Beryllium - August 25, 2016
- What is “Model Building”? - August 18, 2016
- Respecting your “Elders” - June 17, 2016
Something that didn’t make it into the post: I didn’t include a discussion of what the muon conversion experiments really do to get things to work. I realized later that this may answer a few questions that could come up.
A few key points: one source of background comes from pion capture on the aluminum target. In order to remove these, the experiment makes use of the fact that the pions tend to decay more quickly than the muons. By using a pulsed beam, they are able to take measurements during the times when the target contains very few pionic-atoms.
This pulsed beam strategy also sets the choice of aluminum as a target material. In addition to the need for a non-radioactive, sufficiently malleable target, the nucleus cannot be too large or else the probability of muon capture increases and reduces the muon lifetime so much that the muon events are hard to separate from the pion events.
(I thank W. Molzon for discussing this with me after the post.)